© Sashkin/ shutterstock
MOTIVATION
Quantum technology with optical fibers
Optical fibers are the backbone of today’s globally networked communications infrastructure. Hence, it is plausible that this infrastructure will also be used by future fiber-based quantum communication. This requires strong coupling of single photons to controlled quantum systems, which can be achieved with fiber resonators.
Optical fibers also enable the realization of key components such as optical filters and spectrometers for future fiber-based photonic technologies. In this context, miniaturization (made possible by fiber-optic components) brings great advantages in stability, robustness, integration density and thus application potential. Finally, the distribution of frequency standards from optical atomic clocks with an accuracy of up to 10^(-18) using optical fibers will create a new foundation for networked precision metrology and quantum sensing.
Procedures & Equipment
Products
Applications
Research Groups
Publications
Funding
The Fiber Lab at the University of Bonn develops fiber-optic components for quantum technologies.
PROCEDURES AND EQUIPMENT
Micro-machining of fiber end faces by CO2 laser ablation for fabrication of miniaturized optical fiber resonators.
3D microlithography system (Photonic Professional GT by Nanoscribe GmbH) for the production of dielectric optical microstructures and masks for patterned metallization directly on optical fibers.
Fiber cleaver and splicer for highest-precision requirements.
Systems for vapor deposition of metal layers, wire bonding, plasma cleaning and ashing, and fiber profilometers.
PRODUCTS
Fiber mirrors
The Fiber Lab routinely produces concave fiber mirrors on the end faces of single- and multi-mode optical fibers (Fig. 1). The shape of the mirrors is precisely measured using an interference microscope (Fig. 2). After coating with highly reflective dielectric mirrors, fiber resonators are fabricated.
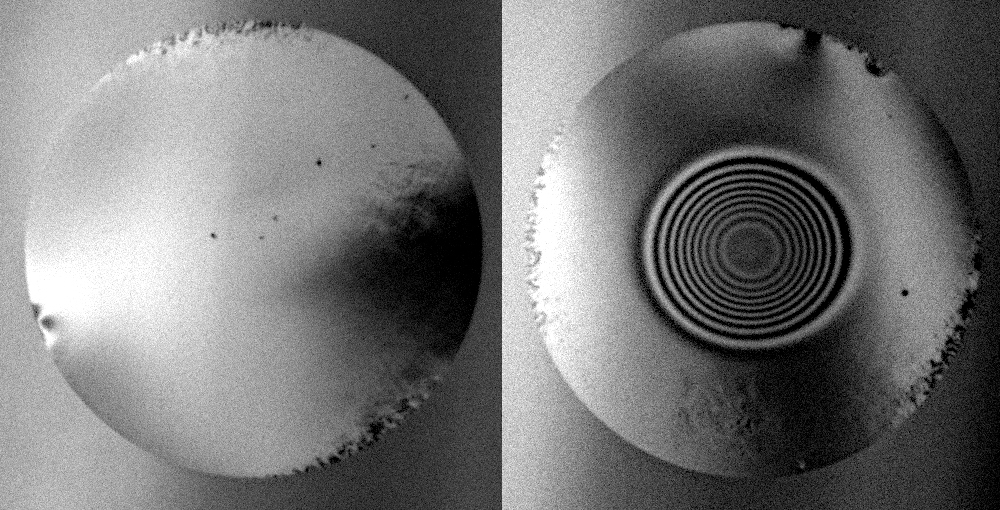
Fig. 1: End face of an optical glass fiber (diameter 125 µm) before (left-hand image) and after (right-hand image) creation of a concave mirror surface by laser ablation. Image produced by interference microscope.
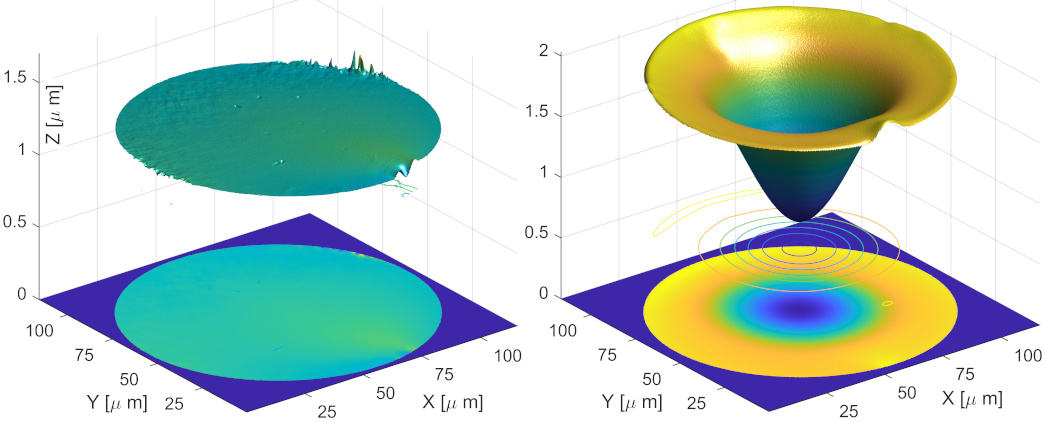
Fig. 2: High-resolution topography of the end face of an optical fiber after separation (“cleaving”, left) and after forming a concave mirror surface by laser ablation (right). Note the vertically magnified image, in reality the cleaving is very shallow.
Optical fiber resonators
Fiber resonators have very small mode volumes (length ~100 µm, radius ~5 µm) with a finesse of up to 100.000 [1]. The small construction volume (Fig. 3) allows for a high passive stability as well as miniaturization and integration into photonic systems. Fiber resonators thus serve as a technical basis for, e.g., quantum memories based on atoms and ions, as well as for filtering and spectroscopy applications [1]. Their coupling efficiency can be increased by combining fibers of different types (Fig. 4).

Fig. 3: Two fibers, machined and mirrored at their ends, are inserted into a glass ferrule to form a very compact monolithic fiber resonator (1-cent coin shown for size comparison).
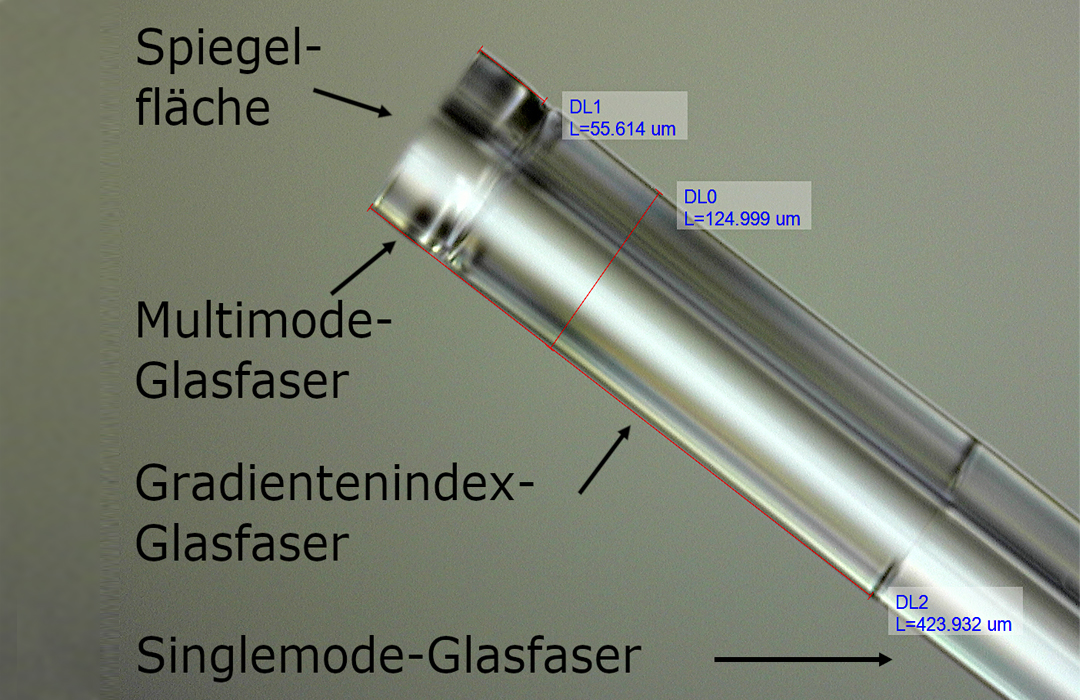
Fig. 4: Here, short pieces of graded-index and multimode fibers, only fractions of a millimeter long, have been spliced onto a single-mode fiber. Fiber lengths deviate from the specified values by less than 5μm. With this setup, mode matching between fiber resonator and singlemode fiber can be significantly improved, maximizing optical coupling and decoupling efficiency.
Metallic micro-electrodes and -antennas on fiber devices
The functionality of optical fiber resonators can be further enhanced by outfitting the fiber end faces and the sides of the fibers with metallic conductors and electrodes. For example, this could be used to integrate the electrodes of an ion trap directly into the optical fiber resonator (Fig. 5). Another potential application is the implementation of RF and microwave near-field antennas, which allow for particularly efficient manipulation of atomic quantum systems using magnetic fields.
While the required minimum structural dimensions of a few micrometers do not pose technical challenge by themselves, current lithography techniques, e.g. electron-beam lithography, are unfortunately not directly applicable since they are usually designed for planar substrates. In addition, one would like to avoid contamination of the highly reflective mirror layers by photoresist residues. The Fiber Lab is developing a microstructuring process specially adapted to the fiber geometry. For this purpose, a special polymer sleeve is first printed using a laser-based 3D microlithography system (in principle a 3D printer for very fine structures, Fig. 6). Then the optical fiber is inserted into the sleeve and installed in a vapor deposition system for metals (mostly gold or copper). The openings in the sleeve define the metallized areas on the optical fiber during the vapor deposition process. In the final step, the polymer sleeve is removed with a micromanipulator after vapor deposition.
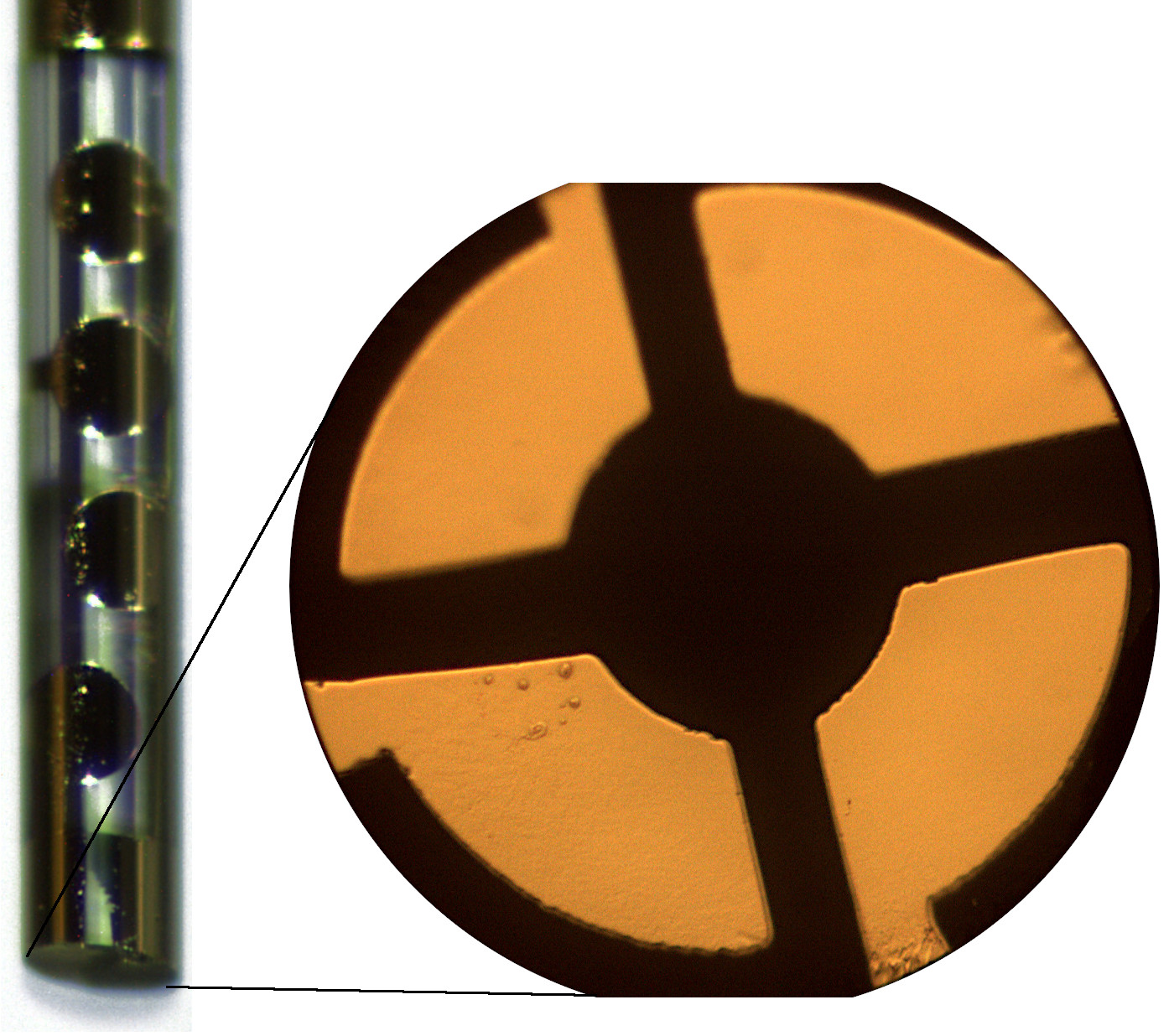
Fig. 5: Four gold electrodes on the end face of an optical fiber. The electrodes were fabricated using a three-dimensional polymer mask and are electrically contacted via the cylindrical cladding surface of the fiber.
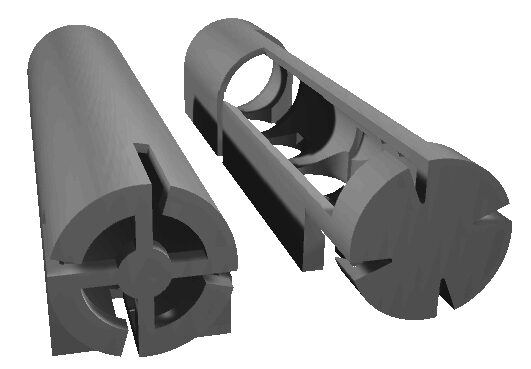
Fig. 6: Design of a three-dimensional polymer mask for vapor deposition of four gold electrodes on the front surface and connection pads on the cladding surface of the fiber.
APPLICATIONS
Fiber-based quantum communications
Privacy of data transmission and protection against hacker attacks are currently achieved by means of encryption algorithms whose secure nature cannot be proven and might even be broken in the future using quantum computers. Quantum communication offers a paradigm shift from algorithmically to physically secured data transmission. Quantum communication can be realized by transmitting photons, in which quantum states are encoded, over optical fibers. However, quantum states cannot be amplified, so today only distances of up to about 100 km can be covered. Hence, future national and Europe-wide quantum networks require so-called quantum repeaters [2]: Intermediate nodes equipped with quantum memories and quantum operations that can generate quantum entanglement between distant nodes, as a resource for quantum communication.
Photonic quantum states (“flying qubits” on the fibers) will be transferred to material qubits (atoms or artificial atoms) at the nodes of future quantum networks for storage. This will require devices such as optical resonators, which will ideally be integrated directly into the fiber links [1].
Networking quantum computers with optical fibers
Single stored ions currently represent one of the two most promising platforms for quantum computing. In particular, long coherence times and the resulting possibility to perform long complex quantum operations make them attractive. A key technology is the networking of quantum computers using light, analogous to fiber-based communication in conventional information processing. Here, fiber-based optical resonators play an important part, since they can be integrated directly into ion traps – the processors of a quantum computer – and enable efficient coupling of the ion trap to fiber networks. The miniaturization of the optical resonators plays a central role, since large resonator structures can interfere with the sensitive qubits in the ion trap (potentially resulting in a total breakdown). The first integration of fiber-based resonators into an ion trap [3] has thus opened a new door to the networking of quantum computers. Ongoing development of fiber resonators towards new wavelength ranges (from ultraviolet to infrared), improved surface coatings, and positioning accuracy will further broaden the range of applicability in the future..
Optical filters and spectrometers
Fiber resonators are very well suited as integrated and narrow-band optical filters. Via the cavity ring-down method, they can also be used as spectrometers with extremely compact design.
RESEARCH GROUPS
Research Group Nonlinear Quantum Optics
Prof. Dr. Sebastian Hofferberth,
Institute of Applied Physics, University of Bonn
Research Group Nanophotonics
Prof. Dr. Stefan Linden,
Physikalisches Institut, University of Bonn
Research Group Experimental Quantum Physics
Prof. Dr. Michael Köhl,
Physikalisches Institut, University of Bonn
PUBLICATIONS
[1] J. Gallego, S. Ghosh, S. K. Alavi, W. Alt, M. Martinez-Dorantes, D. Meschede,
High-finesse fiber Fabry-Perot cavities: stabilization and mode matching analysis
Applied Physics B 122, 47 (2016)
[2] C. Becher, D. Meschede, P. Michler, R. Werner,
Sichere Kommunikation per Quantenrepeater
Physik in unserer Zeit 47, Heft 1, 20 (2016), Verlag Wiley-VCH
[3] M. Steiner, H. M. Meyer, C. Deutsch, J. Reichel, M. Köhl:
Single ion coupled to an optical fiber cavity
Phys. Rev. Lett. 110, 043003 (2013);
see also the Physics Focus story: Optical Fibers Illuminate Single Ion
T. F. Langerfeld, H. M. Meyer, and M. Köhl:
Correlated-photon-pair emission from a cw-pumped Fabry-Perot microcavity
Phys. Rev. A 97, 023822 (2018)
J. Schmitz, H. Meyer, M. Köhl:
Ultraviolet Fabry-Perot cavity with stable finesse under ultrahigh vacuum conditions
Rev. Sci. Instr. 90, 063102 (2019)
F. Rönchen, T. F. Langerfeld and M. Köhl
Correlated photon-pair generation in a liquid-filled microcavity
New J. Phys. 21, 123037 (2019)
P. Kobel, M. Breyer, M. Köhl
Deterministic spin-photon entanglement from a trapped ion in a fiber Fabry-Perot cavity
npj Quantum Information, 7, 6 (2021)
C. Saavedra, D. Pandey, W. Alt, H. Pfeifer, D.Meschede
Tunable fiber Fabry-Perot cavities with high passive stability
Opt. Express 29, 974-982 (2021)
H. Pfeifer, L. Ratschbacher, J. Gallego, C. Saavedra, A. Faßbender, A. von Haaren, W. Alt, S. Hofferberth, M. Köhl, S. Linden, D. Meschede
Achievements and perspectives of optical fiber Fabry–Perot cavities
Appl. Phys. B 128, 29 (2022)
E. Uruñuela, M. Ammenwerth, P. Malik, L. Ahlheit, H. Pfeifer, W. Alt, D. Meschede
Raman imaging of atoms inside a high-bandwidth cavity
Phys. Rev. A 105, 043321 (2022)
A. Ferreri, H. Pfeifer, F. K. Wilhelm, S. Hofferberth, D. E. Bruschi
Interplay between optomechanics and the dynamical Casimir effect
Phys. Rev. A 106, 033502 (2022)
C. Saavedra, D. Pandey, W. Alt, D. Meschede, H. Pfeifer
Spectroscopic Gas Sensor Based on a Fiber Fabry-Perot Cavity
Phys. Rev. Applied 18, 044039 (2022)
L.-M. Needham, C. Saavedra, J. K. Rasch, D. Sole-Barber, B. S. Schweitzer, A. J. Fairhall, C. H. Vollbrecht, B. Mehlenbacher, Z. Zhang, L. Tenbrake, H. Pfeifer, E. R. Chapman, R. H. Goldsmith
Label-free observation of individual solution phase molecules
bioRxiv 2023.03.24.534170 (2023)
L. Tenbrake, A. Faßbender, S. Hofferberth, S. Linden, H. Pfeifer
Direct laser-written optomechanical membranes in fiber Fabry-Perot cavities
Nature Commun. 15, 209 (2024)
K. Krzempek, P. Jaworski, L. Tenbrake, F. Giefer, D. Meschede, S. Hofferberth, H. Pfeifer
Photothermal gas detection using a miniaturized fiber Fabry-Perot cavity
Sens. Act. B 401, 135040 (2024)
S. Gohlke, T. F. Langerfeld, A. Bergschneider, M. Köhl
Coupled high-finesse optical Fabry-Perot microcavities
Phys. Rev. A 109, L011501 (2024)